Richard Feynman knew a thing or two about biology. He is reported to have said, “Everything that living things do can be understood in terms of the jigglings and wigglings of atoms.” That is undoubtedly true, but until very recently almost everything we knew about biological processes at the molecular scale was derived from static images. Now, however, the new crystallography technique of X-ray free-electron laser (XFEL) spectroscopy is enabling structural biologists to take snapshots of atoms “jiggling and wiggling” through complex molecular processes and assemble them into movies.
A step change in the methodology for X-ray crystallography and other structural sciences took place about half a century ago with the advent of synchrotrons as sources of powerful X-ray beams. There are now about 70 synchrotron sources in near constant use worldwide, forming an invaluable research resource for physics, chemistry, and materials science as well as biology. The XFEL represents the next such step change. Tiny crystals—far too small to be used in conventional crystallography—are hit by regular pulses of X-rays powerful enough to pass through steel. Not surprisingly, the crystals explode, but the pulses used are so short that they outrun radiation damage, and usable diffraction data can be obtained in the tiny fraction of a second before the explosion occurs. This has been termed “diffraction before destruction.”
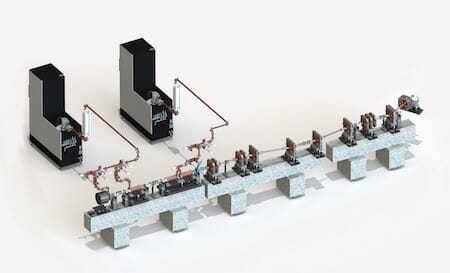
The free-electron laser itself is not particularly new; it was invented by John Madey at Stanford University in 1971. It involves accelerating electrons to speeds near that of light and directing them through a “wiggler”—most often a series of magnets—to change their direction. A resonance condition occurs when the X-ray wavelength equals the wiggle period. The consequent change in the electrons’ acceleration generates a high-intensity beam of electromagnetic radiation. Mirrors or an external laser further intensify the radiation, make it coherent, and tune it precisely to a single frequency anywhere on the electromagnetic spectrum from the microwave to the X-ray band.
The world’s first XFEL to produce “hard” x rays for crystallography opened in 2009 at the SLAC National Accelerator Laboratory in California. Since then, XFEL facilities have opened in Germany, Japan, and South Korea, and a fifth will open in Switzerland soon. In collaboration with MIT, Arizona State University is developing a compact XFEL (CXFEL), now under construction in its new Biodesign Institute building. Arizona State is the largest component of NSF’s BioXFEL Science and Technology Center, established in 2013 and led by scientific director John Spence with other collaborators. Its 10-year NSF grant to a consortium of eight universities is enabling it to pioneer the use of XFELs for biology. The compact X-ray free-electron laser under construction at Arizona State University. The L-shaped boxes in this artist’s impression are the two 6 MW klystron power sources. Although the beamline is just 10 m long, it will deliver fully coherent sub-fs pulses of 0.5–8 keV x rays at a repetition rate of 1 kHz. (Image courtesy of Arizona State University.)
Spence and coprincipal investigator Petra Fromme presented the state of the art in XFEL technology at a recent conference in Liverpool, UK, held to celebrate 50 years of synchrotron radiation research, and explained the many advantages of the technique. Whereas conventional X-ray crystallography of proteins and other large molecules requires relatively large crystals that are difficult to grow, those used in the XFEL can be several orders of magnitude smaller; they grow much more easily. What’s more, since damage is outrun, it is no longer necessary to freeze crystals to avoid radiation damage. With the femtosecond X-ray pulses of the free-electron laser, molecular machines can be observed in action at physiological temperatures.
The electron accelerator for the original XFEL at Stanford was two miles long. In contrast, the XFEL under development at Arizona State is a compact machine that fits into a large room; the university funded it and hired Bill Graves from MIT as faculty to design and build it. It uses an IR laser running antiparallel to the electron beam as its wiggler, producing a highly monochromatic pulsed beam of intense x rays. The pulses can be even shorter than those generated by older, full-scale XFEL machines: almost down to the attosecond rather than femtosecond scale. This is bound to expand the range of systems that can be studied, but its greatest advantage is likely to be an economic one. Access to XFEL beam time is currently hotly contested, but with the cost of CXFELs plummeting, more machines may soon be built in Australia, Denmark, Germany, and the UK to ease the logjam.
Fromme, director of the Center for Applied Structural Discovery, which will host the CXFEL, told the Liverpool meeting how XFELs will enable the Arizona State team to understand a “holy grail of structural biology”: the dynamic structure of the large, membrane-bound plant enzymes known as photosystems and the molecular process of photosynthesis. The new attosecond-scale XFELs will allow her group to study the ultrafast process of water splitting and oxygen evolution, catalyzed by Photosystem II, at high temporal and spatial resolution. Molecular movies have already been made of a molecular “switch” for turning off protein synthesis and of the process by which beta-lactamase enzymes inactivate penicillin-like antibiotics. With antibiotic resistance becoming a global threat to rival climate change, we need to develop novel tools to fully understand and combat this process. The XFEL is one of these.
Source: Physics Today